
CONTRACTION
OF
GLYCERINATED
MUSCLE
FIBERS
AS
A
FUNCTION
OF
THE
ATP
CONCENTRATION
ROGER
COOKE
AND
WILLIAM
BIALEK,
Department
of
Biochemistry
and
Biophysics
and
Cardiovascular
Research
Institute,
University
of
California,
San
Francisco,
California
94143
U.S.A.
ABSTRACT
We
have
measured
the
force-velocity
curves
of
glycerinated
rabbit
psoas
fibers
over
a
range
of
ATP
concentration
from
2.5
gM
to 5
mM.
As
the
ATP
concentration
is
increased,
the
isometric
tension
increases
to
a
maximum
around
50
.IM,
then
decreases
to
a
plateau
at
70%
of
the
maximum
by
1
mM
ATP.
At
low
ATP
concentrations
the
maximum
velocity
of
contraction
is
low
and
increases
with
increasing
ATP,
reaching
a
plateau
at
-2
lengths
per
second
by
1
mM
ATP.
Our
studies
suggest
that
the
binding
of
ATP
dissociates
the
myosin
head
from
actin
in
the
contracting
muscle,
a
reaction
similar
to
that
seen
in
solution.
We
have
constructed
models
of
the
actin-myosin-nucleotide
interactions
based
on
a
kinetic
scheme
derived
from
solution
studies.
The
fit
of
these
models
to
the
data
shows
that
the
rates
of
some
reactions
in
the
fiber
must
be
considerably
different
from
the
rates
of
the
analogous
reactions
in
solution.
The
data
is
best
fit
by
models
in
which
head
attachment
occurs
rapidly
at
the
beginning
of
a
power
stroke,
head
detachment
occurs
rapidly
at
the
end
of
the
power
stroke,
and
the
force
produced
by
a
myosin
head
in
a
power
stroke
is
independent
of
velocity.
INTRODUCTION
The
force
of
contraction
in
a
muscle
cell
is
generated
by
the
cyclic
interaction
of
two
proteins,
actin
and
myosin.
The
free
energy
that
drives
this
reaction
is
derived
from
the
hydrolysis
of
ATP.
Myosin
has
two
heads
that
extend
radially
from
the
thick
filament
and
that
interact
with
the
actin
(or
thin)
filament.
It
is
now
thought
that
in
a
contracting
muscle
the
myosin
heads
attach
to
the
actin,
execute
a
power
stroke,
and
then
detach.
The
interactions
of
actin,
myosin
(or
myosin
heads),
and
ATP
have
been
studied
extensively
in
solution
and
a
number
of
intermediate
states
and
the
transition
rates
between
them
have been
identified
(for
review
see
Lymn
1974,
1975).
When
ATP
binds
to
the
myosin
nucleotide
site
of
an
actomyosin
complex,
the
myosin
is
rapidly
released
from
the
actin
filament
(Lymn
and
Taylor,
1971;
Sleep
and
Taylor,
1976).
Myosin
splits
the
ATP
and
the
myosin-products
complex
rebinds
to
actin,
followed
by
product
release
(Finlayson
et
al.,
1969).
It
is
assumed
that
a
similar
cycle
of
events
occurs
in
the
muscle
fiber;
however,
the
Note
added
in
proof:
Ferenczi
et
al.
(
1979)
have
recently
reported
force-velocity
curves
for
skinned
frog
muscle
in
5
and
0.3
mM
ATP.
Their
results
are
qualitatively
similar
to
those
reported
here.
They
find
that
at
0.3
mM
ATP
Vm
is
0.63
lengths/s,
which
is
approximately
a
factor
of
two
slower
than
we
found
for
rabbit
muscle.
The
rate
constant
for
dissociation
of
frog
actomyosin
by
ATP
is
also
approximately
two
times
slower
than
that
for
rabbit
actomyosin
(Ferenczi
et
al.,
1978;
White
and
Taylor,
1976;
Sleep
and
Taylor,
1976).
Thus
the
rate
constant
for
the
dissociation
of
actomyosin
by
ATP
appears
related
to
Vm
measured
at
low
ATP
as
predicted
by
the
model
presented
above.
BIOPHYS.
J.
©
Biophysical
Society
.
0006-3495/79/11/241/18
$1.00
241
Volume
28
November
1979
241-258

kinetic
methods
that
have been
used
to
establish
this
cycle
in
solution
are
difficult
or
impossible
to
apply
to
the
organized
filament
array
of
the
fiber.
The
physiological
properties
of
muscle
fibers
have been
studied
through
measurements
of
force,
contraction
velocity,
heat
output,
and
ATPase
activity
(A.
V.
Hill,
1938,
1964;
Curtin
et
al.,
1974;
Edman
and
Hwang,
1977).
More
recently,
the
transient
responses
of
fibers
to
changes
in
length
or
tension
have
given
additional
data
(Huxley
and
Simmons,
1971;
Civan
and
Podolsky,
1966).
A
number
of
theories
have
been
constructed
that
attempt
to
explain
the
characteristics
of
muscle
fibers
in
terms
of
the
kinetics
of
the
myosin
cross-bridges.
A.
F.
Huxley
(1957)
was
the
first
to
propose
such
a
theory
and
subsequent
theories
have
been
proposed
by
Podolsky
and
Nolan
(1972),
Huxley
and
Simmons
(1972),
Julian
et
al.
(1974),
and
Borejdo
(1978).'
These
theories
have
characterized
the
cross-bridge-actin
interaction
in
terms
of
rates
for
attachment,
power
stroke
execution,
and
detachment.
They
have
not
explicitly
incorporated
the
interactions
with
nucleotides
that
are
now
well
understood
in
the
solution
studies.
A
formalism
to
incorporate
energetics
and
nucleotide
interactions
has
been
presented
by
Hill
(1974),
Hill
et
al.
(1975),
and
Eisenberg
and
Hill
(1978).
However,
applications
of
this
approach
are
difficult
because
the
cycle
of
the
actomyosin-ATP
interac-
tion
is
complex,
and
rates
measured
in
solution
may
not
apply
in
the
intact
filament
array
of
the
muscle
fiber.
Studies
of
the
mechanical
responses
and
the
energetics
of
intact
fibers
have
not
generated
sufficient
data
to
determine
such
a
scheme.
Data
allowing
one
to
link
biochemical
kinetics
with
force
production
are
required
before
complex
models
of
muscle
contraction
can
be
understood.
By
varying
the
ATP
concentration,
we
vary
the
rate
of
one
reaction
in
the
cycle
of
interactions
that
generates
force,
i.e.,
the
rate
of
the
reaction
in
which
ATP
first
enters
the
cycle.
Thus,
by
recording
force-velocity
relations
at
varying
ATP
concentrations
we
see
how
the
properties
of
the
muscle
depend
upon
the
transition
rate
between
two
biochemical
states,
and
hence
make
one
link
between
the
biochemistry
and
physiology
of
the
system.
This
link
has
allowed
us
to
draw
conclusions
about
the
properties
of
the
nucleotide
interactions
in
the
fiber,
and
these
conclusions
have
led
in
turn
to
a
more
detailed
picture
of
the
general
nature
of
force
production.
METHODS
Thin
strips
of
rabbit
psoas
fibers
(1-2
mm
Diam)
were
dissected
and
tied
to
wooden
sticks.
They
were
incubated
at
0°C
for
12
to
24
h
in
50%
glycerol/50%
0.1
M
KCl,
10
mM
K2HPO4,
5
mM
MgCl2,
2
mM
EGTA,
pH
7.
The
solution
was
changed
and
the
fibers
were
then
stored
at
-
200C.
Fibers
were
used
for
up
to
3
mo.
Storage
did
not
appear
to
affect
their
characteristics.
Fibers
were
dissected
in
the
above
solution
on
a
cold
stage
(temperature,
<0°C).
Single
fibers
or
bundles
of
up
to
three
fibers
(2-4
mm
long)
were
mounted
on
a
tensiometer
using
the
same
techniques
described
by
Crooks
and
Cooke
(1977)
for
actomyosin
threads.
The
solution
bathing
the
fibers
contained
0.15
M
KCI,
5
mM
MgCl2,
2
mM
EGTA,
1.9
mM
CaC12,
20
mM
N-tris
(hydroxymethyl)
methyl-2-aminoethane
sulfonic
acid,
pH
7,
pCa
-5
(buffer
1).
In
all
experiments
at
10
mM
ATP
the
Mg
concentration
was
increased
to
10
mM
and
the
ionic
strength
was
adjusted
to
that
of
buffer
1
by
decreasing
the
KCI
concentration
and
by
assuming
that
all
the
ATP
was
complexed
to
Mg.
Contraction
was
initiated
by
addition
of
ATP.
Fibers
developed
maximal
tension
within
a
few
seconds,
and
could
maintain
a
stable
tension
for
more
than
5
min.
The
tensiometer
has
been
described
by
Crooks
and
Cooke
'Borejdo,
J.
Personal
communication.
BIOPHYSICAL
JOURNAL
VOLUME
28
1979
242

A
0
C4
_
_
p
0
~~~~~~0
cn
~~~~~~~~~~~~~~~~~~~U)
-4
o
0
20
40
10
20
[Creotine
Kinose]
,u
M
[Creotine
Pi]
mM
FIGURE
1
(A)
The
maximum
velocity
of
contraction
in
lengths/second
(1/s)
is
shown
as
a
function
of
the
concentration
of
creatine
kinase
added
to
the
medium
(buffer
1
plus
20
,M
ATP
and
20
mM
creatine
phosphate).
(B)
The
velocity
of
contraction
is
shown
as
a
function
of
the
creatine
phosphate.
The
medium
contained
buffer
1
plus
20
,M
ATP
and
30
,gM
creatine
kinase.
The
temperature
was
10
±
0.50C.
(1977).
It
was
operated
in
an
isometric
mode
until
the
tension
stabilized
after
addition
of
ATP;
the
tension
was
then
dropped
rapidly
(5
ms)
and
the
length
of
the
fiber
recorded
as
a
function
of
time.
We
found
that
the
fibers
gave
reproducible
velocity
traces
for
approximately
five
contractions.
The
fiber
then
began
to
display
a
large
initial
drop
in
length
after
the
tension
step
(>
12
nm/half
sarcomere)
and
the
velocity
decreased
as
the
contraction
proceeded.
Thus,
only
the
first
2-5
contractions
per
fiber
were
used,
and
the
force-velocity
curves
shown
are
averages
from
several
fibers.
The
diameters
of
the
fibers
were
measured
with
a
microscope
at
several
positions
along
the
fiber,
and
the
values
averaged.
All
force
measurements
were
made
at
10°C.
Creatine
kinase
was
bought
from
Boehringer
Mannheim
Biochemi-
cals
(Indianapolis,
Ind.).
RESULTS
The
diffusion
of
ATP
into
a
muscle
fiber
is
slow
compared
with
its
rate
of
hydrolysis;
thus
the
level
of
ATP
must
be
maintained
by
a
feeder
system.
We
choose
to
use
the
creatine
kinase-creatine
phosphate
system
because
it
operates
in
the
living
fiber.
The
velocity
of
contraction
was
much
more
sensitive
to
the
level
of
ATP
than
was
the
isometric
tension;
thus
the
feeder
system
was
characterized
by
studying
the
velocity
of
contraction
as
a
function
of
the
concentrations
of
creatine
kinase
and
creatine
phosphate.
The
results
are
shown
in
Figs.
1
A
and
B.
At
a
low
level
of
ATP
(20
,uM),
the
velocity
of
contraction
was
very
low
in
the
absence
of
a
feeder
system.
As
creatine
phosphate
was
raised
(in
an
excess
of
creatine
kinase),
the
contraction
velocity
increased
and
then
reached
a
plateau.
A
similar
effect
was
seen
for
variation
of
creatine
kinase.
The
increase
in
the
contraction
velocity
that
occurs
upon
the
addition
of
creatine
phosphate
in
the
absence
of
creatine
kinase
indicates
that
some
creatine
kinase
had
remained
bound
to
the
fiber
in
spite
of
the
glycerination
procedure.
Subsequent
studies
were
performed
with
levels
of
creatine
kinase
and
creatine
phosphate
that
were
more
than
sufficient
to
saturate
the
effect
on
the
contraction
velocity.
Fig.
2
shows
the
isometric
tension
as
a
function
of
ATP
concentration.
In
the
absence
of
added
ATP
the
fiber
exerted
a
low
tension
of
about
0.01
N/mm2
probably
due
to
residual
ATP
in
the
fiber.
As
ATP
was
added,
the
tension rose
to
a
maximum
at
-30-50
,uM
ATP,
COOKE
AND
BIALEK
Contraction
of
Glycerinated
Muscle
Fibers
B
243

0~~~~~~~~~~~
I
0.50
°
0.10
4
0
50
E
0
GD
0.25
0.05-
E~~~~~~~~~~~~~~
0
0
40
80
500
1000
00.5
1.0
[ATPI
jpM
F/
FO
FIGURE
2
FIGURE
3
FIGURE
2
The
isometric
tension
is
plotted
as
a
function
of
the
ATP
concentration.
The
medium
contained
buffer
1
plus
20
mM
creatine
phosphate
and
30
/LM
creatine
kinase.
The
temperature
was
controlled
at
10
±
0.50C.
The
solid
line
represents
a
fit
to
the
data
as
described
in
the
text.
The
isometric
tension
at
5
mM
ATP
(not
shown)
was
85%
of
that
at
1
mM
ATP,
and
at
10
mM
ATP
it
was
81%
of
that
at
1
mM
ATP.
This
drop
was
included
in
the
analysis
of
the
data.
FIGURE
3
Force-velocity
curves
for
different
ATP
concentrations:
10
,uM
(O
),
50
,uM
(-),
100
,uM
(0),
and
500
,uM
(A).
The
conditions
were
the
same
as
in
Fig.
2.
The
solid
lines
represent
a
fit
to
the
data
as
described
in
the
text.
then
decreased
as
ATP
was
raised
to
1
mM.
In
contrast
to
the
isometric
tension,
which
is
not
a
strong
function
of
ATP
concentration
in
the
range
50
4AM
to
1
mM,
the
force-velocity
relation
was
highly
dependent
on
ATP
concentration
up
to
1
mM
ATP,
(Figs.
3
and
5).
To
extrapolate
the
data
to
a
maximum
contraction
velocity
at
F
=
0,
the
force
velocity
curves
were
fit
by
a
linearized
version
of
the
Hill
equation.
Several
fits
are
shown
in
Fig.
4.
The
maximum
contraction
velocity,
obtained
from
plots
such
as
those
shown
in
Fig.
4,
is
shown
as
a
function
of
the
ATP
concentration
in
Fig.
5.
The
presence
of
a
feeder
system
is
required
to
obtain
a
maximum
contraction
velocity
of
2
lengths/s.
In
the
absence
of
the
feeder
system
the
maximum
contraction
velocity
was
-0.7
lengths/s
in
the
presence
of
either
4
or
10
mM
ATP
(Fig.
6).
The
fact
that
both
ATP
concentrations
give
identical
force-velocity
curves
indicates
that
the
build
up
of
ADP
and
not
the
availability
of
ATP
causes
the
decreased
velocities
compared
with
those
obtained
with
a
feeder
system.
DISCUSSION
General
Procedure
The
force-velocity
curves
we
have
measured
provide
constraints
that
help
define
models
of
the
interaction
of
actin,
myosin,
and
nucleotides
in
a
contracting
fiber.
Biochemical
experiments
BIOPHYSICAL
JOURNAL
VOLUME
28
1979
244

-i
"I
c
4)
Z,
.5
0
c
.0
Q
x
'2
F/
Fo
FIGURE
4
0.5
[ATP]
(mM)
FIGURE
5
FIGURE
4
A
fit
of
the
Hill
force-velocity
relation
to
the
data
is
shown
for
two
ATP
concentrations;
(o)
1
mM
and
(-)
100
gM.
The
Hill
equation
(Hill,
1938)
was
plotted
in
the
form:
(I
-
F/Fo)v-'
=
F/Fob
+
a/Fob
where
F,
Fo,
and
v
are
the
force,
isometric
force,
and
velocity
of
contraction,
respectively,
and
a
and
b
are
two
constants
that
define
the
hyperbolic
force-velocity
relationship.
Extrapolation
to
F
=
0
gives
the
maximum
velocity
V,'
=
Pob/a.
As
ATP
is
lowered
the
force
velocity
curves
still
fit
the
hyperbolic
relationship
with
a
constant
value
of
b
and
an
increased
value
of
a.
FIGURE
5
The
maximum
velocity
of
contraction
is
plotted
as
a
function
of
the
ATP
concentration.
The
maximum
contraction
velocity
was
determined
by
fitting
a
linear
form
of
the
Hill
equation
as
shown
in
Fig.
4.
The
error
bars
represent
standard
errors
of
the
mean
from
at
least
5
force-velocity
curves.
0
1.0
-c
~.0.5
0.5
1.0
F/
Fo
FIGURE
6
Force-velocity
curves
at
high
ATP
concentrations
in
the
absence
and
presence
of
an
ATP
regenerating
system.
The
medium
contained
buffer
1
plus
4
mM
ATP
(o);
buffer
I
plus
10
mM
ATP
with
the
ionic
strength
adjusted
as
described
in
Methods
(e);
or
buffer
1
plus
5
mM
ATP
with
30
MAM
creatine
kinase
and
20
mM
creatine
phosphate
(O).
The
upper
curve,
obtained
in
the
presence
of
the
ATP
regenerating
system,
has
been
fit
to
the
data
as
described
in
the
text,
while
the
lower
curve
was
drawn
by
eye.
COOKE
AND
BIALEK
Contraction
of
Glycerinated
Muscle
Fibers
In
-c
c
LI
0
U-
245

have
suggested
a
kinetic
scheme
for
this
interaction
in
solution
(Sleep
and
Taylor,
1976;
White
and
Taylor,
1976;
Chock
et
al.,
1976;
Lymn,
1974,
1975),
and
we
use
this
kinetic
scheme
as
a
starting
point
for
models
of
the
analogous
interaction
in
the
fiber.
Thus,
we
assume
that
force
is
produced
by
a
cyclic
interaction
involving
the
dissociation
of
myosin
and
actin
by
ATP,
the
hydrolysis
of
the
ATP
by myosin
while
dissociated
from
actin,
the
rebinding
of
a
myosin-products
complex
to
the
actin,
followed
by
a
power
stroke.
Recent
evidence
shows
that
the
two
myosin
heads
produce
force
independently
in
actomyosin
threads
(Cooke
and
Franks,
1978),
and
there
is
growing
evidence
that
the
two
heads
of
each
myosin
molecule
have
identical
primary
chain
sequences
(Holt
and
Lowey,
1977;
Schachat
et
al.,
1977).
Thus,
the
most
reasonable
model
involves
the
independent
action
of
each
head
in
the
force-producing
interaction.
We
assume
that
one
ATP
is
split
during
each
cycle.
We
first
fit
the
isometric
tension
as
a
function
of
ATP
concentration
(Fig.
2),
assuming
that
the
cycle
of
events
described
above
occurs
in
a
fiber
but
that
rates
that
involve
an
actomyosin
complex
may
differ
from
those
measured
in
solution.
We
show
that
this
model
can
explain
the
isometric
data.
We
then
use
energetic
relations
to
derive
some
conclusions
from
the
isotonic
data
taken
at
low
ATP
concentrations.
The
conclusions
from
both
the
isotonic
and
isometric
data
are
then
used
to
define
a
simple
model
that
can
explain
all
the
data.
The
Isometric
Model
The
complete
kinetic
scheme,
constructed
from
the
solution
data,
for
the
interaction
of
actin,
myosin,
and
nucleotides,
is
too
complex
to
be
determined
by
the
fiber
data.
Thus
we
simplify
the
kinetic
scheme
to
four
states,
recognizing
that
some
of
these
states
are
composites
of
several
individual
states.
We
ignore
the
back
rate
constants,
so
that
the
rates
can
be
thought
of
as
the
flux
from
one
state
to
the
next.
The
kinetic
scheme
with
which
we
will
fit
the
isometric
data
is
shown
below:
(State
3)
k
(State
4)
A
+
M
*
ATP
A
+
M
*
Pr.
lk23
lk4l
ATP
k12
A-M
A
.
MA
Pr.
(State
2)
(State
1)
Pr
where
A
represents
actin,
M
represents
myosin,
and
Pr
represents
either
ADP
or
ADP
+
P,.
During
an
isometric
contraction
force
is
generated
by
myosin
heads
interacting
with
actins
that
are
distributed
throughout
some
force-producing
region,
and
we
assume
that
the
above
cycle
can
describe
the
interaction
of
all
of
these
heads.
Our
present
goal
is
to
ask
whether
this
BIOPHYSICAL
JOURNAL
VOLUME
28
1979
246

simple
scheme
will
explain
the
curve
of
isometric
tension
vs.
[ATP],
and
if
so,
to
determine
the
rate
constants
of
the
model
by
the
fit
to
the
data.
Since
ATP
is
capable
of
dissociating
the
actomyosin
complex,
it
is
clear
that
there
exists
a
species
of
that
complex
whose
population
tends
to
zero
as
the
ATP
concentration
becomes
large.
The
decrease
in
the
isometric
tension
that
occurs
as
the
ATP
concentration
is
increased
above
50
,uM
can
be
explained
if
this
dissociable
species,
which
we
write
as
A.
M.,
is
force
producing.
The
tension
does
not
fall
to
zero
as
the
ATP
concentration
is
increased,
however,
so
that
the
data
demand
the
existence
of
a
second
force-producing
species
of
the
actomyosin
complex
that
cannot
be
dissociated
by
ATP.
While
this
undissociable
species
may
be
constructed
by
having
the
myosin
head
assume
an
undissociable
conformation
after
a
rapid
release
of
products,
it
seems
more
natural
to
allow
the
products
to
remain
bound
in
the
state
A
.
M
*
Pr,
thus
protecting
the
head
from
dissociation
by
ATP
as
the
data
require.
Thus,
both
the
states
in
which
actin
and
myosin
are
complexed
in
the
above
scheme
must
generate
force.
The
isometric
tension
will
be
proportional
to
the
number
of
myosin
heads
capable
of
participating
in
the
cyclic
interaction
with
actin
and
to
the
expected
force
produced
in
the
cycle.
The
expected
force
produced
in
the
cycle
is
just
F
=
fPi.,
wheref
is
the
force
produced
by
the
state
i
and
pi
is
the
probability
of
being
in
the
state
i(I
Pi
=
1).
We
shall
assume
that
only
the
two
attached
states
produce
force,
and
that
they
produce
equal
force
(the
assumption
of
equal
force
will
be
discussed
later).
Hence:
F(O,
T)
=
Fa(PA.M.Pr
+
PA-M)
(probability
of
cyclic
interaction),
(1)
where
F(O,
T)
is
the
tension
at
zero
velocity
and
[ATP]
=
T.
One
can
write
down
four
differential
equations
that
describe
the
rate
of
change
of
the
population
of
each
of
the
four
states
in
terms
of
the
rate
constants,
the
populations
of
the
states,
and
the
concentration
of
ATP.
At
steady
state
the
rate
of
change
of
each
state
is
set
equal
to
zero,
and
the
equations
solved
to
give
PA.M.PT
and
PA.M.
All
factors
affecting
the
probability
of
interaction
that
do
not
depend
on
[ATP]
may
be
absorbed
into
the
constant
Fa.
At
very
low
[ATP],
the
tension
is
low
because
few
myosin
heads
are
participating
in
a
contractile
cycle.
This
is
a
complex
phenomenon
that
represents
a
balance
between
the
rate
at
which
ATP
transfers
heads
into
force-generating
states
and
the
rate
of
relaxation
of
these
heads
back
to
states
that
are
not
generating
a
net
force.
A
description
of
this
process
goes
beyond
our
present
scope
and
we
describe
the
low
tensions
at
low
[ATP]
phenomenologically
by
an
apparent
Michaelis
Menten
constant
Km.
The
isometric
tension
is
given
by:
k12
+
k23TT
F(O,
T)
=
Fak+kT+kkT
K+
T
(2)
where
r
=
k3'
+
k4'
represents
the
average
time
spent
dissociated.
Eq.
2
was
fit
to
the
isometric
tension
as
a
function
of
the
ATP
concentration
by
a
nonlinear
least-squares
method
by
using
standard
programs
on
a
Hewlett-Packard
9825
desk-top
calculator
(Hewlett-Packard
Co.,
Palo
Alto,
Calif.).
The
equation
provides
a
good
fit
to
the
data,
as
shown
in
Fig.
2.
Km
is
determined
by
the
increase
in
tension
at
low
ATP
concentrations,
and
is
found
to
be
2.3
±
0.5
,uM.
The
first
factor
in
Eq.
2
can
be
reduced
to
two
variables
by
dividing
both
denominator
and
numerator
by
any
one
of
the
variables
(e.g.,
k12)
and
the
portion
of
the
curve
at
higher
ATP
concentrations
in
Fig.
2
contains
sufficient
COOKE
AND
BIALEK
Contraction
of
Glycerinated
Muscle
Fibers
247

information
to
determine
these
two
variables.
The
data
thus
fix
the
ratios
of
the
rate
constants
k12,
k23,
and
r-I:
k23/kl2
=
4.2
±
1.1
x
103
M-1;
kl2r
=
0.94
±
0.12.
(3)
The
fit
to
the
data
provides
narrow
constraints
on
the
parameters,
as
shown
above,
but
does
not
contain
sufficient
information
to
uniquely
determine
all
the
rates
in
the
model.
The
absolute
values
of
the
above
parameters
can
be
determined
if
one
knows
the
rate
at
which
heads
complete
an
entire
cycle.
This
rate
is
the
ATPase
rate
for
the
whole
muscle
divided
by
the
fraction
of
heads,
fo,
that
are
actively
participating
in
a
tension-generating
cycle.
The
factorfo
must
be
introduced
because
we
do
not
know
the
fraction
of
heads
that
will
have
an
actin
site
with
which
an
ATP
splitting
interaction
can
occur
during
an
isometric
contraction,
e.g.,
in
the
model
presented
in
Fig.
8,fo
is
(X2
-
X1)/L.
The
cycle
time
is
easily
evaluated
to
give
the
isometric
ATPase
at
high
ATP,
Rmax:
Rmax
=f0[k2'
+
-T]
(4)
The
value
of the
isometric
ATPase
has
been
measured
by
several
workers
(Takashi
and
Putnam,
1979;
Steiger,
1977;
Arata
et
al.,
1977)
and
we
take
an
average
value
to
be
-0.3
s-'
(per
head
at
1
0°C).
The
value
offo
cannot
be
determined
exactly
but
can
be
constrained
to
lie
within
a
certain
range.
It
must
be
<
1,
and
a
lower
limit
can
be
estimated
from
the
fact
that,
as
the
value
decreases,
fewer
heads
are
attached
and
thus
each
must
be
generating
more
tension.
Taking
0.2
N/mm2
as
the
isometric
tension
and
assuming
that
each
head
exerts
appreciable
force
over
12
nm
(Barden
and
Mason,
1978),
and
that
it
cannot
perform
more
work
than
that
available
from
one
ATP
(-50
kJ/mol,
Curtin
et
al.,
1974),
one
calculates
that
more
than
17%
of
the
heads
must
produce
force
simultaneously.
Of
those
heads
that
can
participate
in
the
cycle,
(1
+
k12
r)-'
=
0.52
are
attached
at
high
[ATP],
and
thus,fo
is
approximately
>0.3.
Although
this
calculation
is
not
an
exact
one,
it
provides
a
rationale
for
a
lower
limit
on
the
number
of
attached
heads
and
establishes
a
rough
estimate
of
the
actual
limit.
We
note
that
this
argument
can
be
reversed
to
yield
an
upper
bound
forf0.
Since
we
know
that
the
muscle
is
capable
of
operating
at
-50%
efficiency,
the
work
per
head
must
be
at
least
half
of
the
ATP
energy.
Thus,fo
is
<
0.7.
By
using
a
range
(0.7
>
fo
>
0.3),
the
kinetic
parameters
of
the
model
are
found
to
be:
0.8
<kl2<1.8
s-'
3.6
x
103
<k23<7.6
x
103
M
-'s
0.8
<r-
<1.9
s
-.
(5)
The
value
of
k12
is
lower
than
that
which
has
been
measured
indirectly
in
solution
(100
s-'
[Lymn,
1974]).
The
slow
release
of
products
insures
that
at
high
[ATP],
the
head
will
remain
attached
for
sufficient
time
and
thus
the
"tension
cost"
will
be
low.
The
value
of
k23
is
about
two
orders
of
magnitude
lower
than
that
measured
in
solution
(White
and
Taylor,
1976).
The
value
of
k12
r
determines
the
fraction
of
attached
heads
for
those
heads
that
are
interacting
with
actin
and
producing
force.
This
fraction
depends
on
the
assumption
that
A.
M
and
A-
M
-
Pr
produce
the
same
force.
This
assumption
is
certainly
arbitrary
and
the
forces
generated
could
be
different.
If
these
forces
differed
by
more
than
a
factor
of
three
a
BIOPHYSICAL
JOURNAL
VOLUME
28
1979
248

satisfactory
fit
to
the
fraction
of
attached
heads
at
high
ATP
could
not
be
obtained.
Variations
of
less
magnitude
will
change
primarily
the
factor
k,2r
and
estimates
of
fo
but
will
not
affect
the
overall
conclusions
drawn
from
this
model.
Our
model
also
predicts
the
dependence
of
the
isometric
ATPase
on
the
ATP
concentra-
tion.
Those
myosin
heads
that
are
participating
in
a
contractile
cycle
will
have
an
isometric
ATPase
that
is
given
by:
R(T)
=
Rmax
;
where
Kap
=
l
2/k23
(6)
maKap
±T
ap
1+
k12r
(6
Above
approximately
20
,uM
ATP
the
probability
of
participating
in
a
contractile
cycle
is
independent
of
the
ATP
concentration
and
Rmax
is
thus
a
constant.
The
value
of
Kapp,
and,
hence,
the
dependence
of
the
isometric
ATPase
rate
on
ATP
concentration
is
predicted
by
the
fit
of
the
model
to
the
data
of
Fig.
2,
and
has
a
value
of
125
±
30
,uM.
This
is
much
higher
than
the
Km
for
acto-myosin
ATPase
in
solution,
-6
,uM
(Moos,
1972);
or
myofibril
ATPase,
-15
,uM
(Goodno,
1978).
This
prediction
was
recently
confirmed
by
Takashi
and
Putnam
(1979)
in
a
direct
measurement
of
the
ATPase
of
an
isometrically
contracting
fiber.
They
observe
that
half
saturation
is
reached
at
-
150
,uM,
an
order
of
magnitude
above
estimates
from
solution
studies
and
in
excellent
agreement
with
our
prediction.
Isotonic
Contractions
The
first
theory
of
myosin
cross-bridge
kinetics
put
forward
to
explain
the
characteristics
of
muscle
contraction
was
proposed
by
A.
F.
Huxley
in
1957.
In
this
model
the
myosin
is
dissociated
rapidly
at
the
end
of
a
power
stroke
with
a
rate
g2
in
a
region
in
which
the
head
is
exerting
a
negative
tension
(i.e.,
a
tension
that
opposes
contraction).
The
most
straightforward
modification
of
this
theory
that
will
allow
it
to
describe
data
at
various
ATP
concentrations
is
to
make
g2
dependent
on
the
ATP
concentration.
As
[ATP]
decreases,
corresponding
to
lower
values
of
g2,
the
maximum
contraction
velocity
in
this
model
decreases
in
a
manner
similar
to
that
observed.
However,
the
shapes
of
the
curves
are
distinctly
different
from
the
experimen-
tal
ones,
as
shown
in
Fig.
7.
At
low
ATP
concentrations
the
theoretical
curve
is
concave
downward
with
a
negative
second
derivative,
while
the
observed
curve
is
concave
upward
with
a
positive
second
derivative.
Furthermore,
it
can
be
shown
that
modifications
of
the
force-distance
relation
in
the
region
of
negative
force
cannot
solve
this
problem.
We
thus
conclude
that
dissociation
of
the
head
by
ATP
is
not
significant
in
the
region
in
which
negative
work
is
done.
We
have
found
that
the
experimental
data
can
be
fit
by
a
model
in
which
the
myosin
head
is
dissociated
by
ATP
in
a
region
that
preceeds
the
region
in
which
negative
work
is
performed.
We
may
assume
(quite
generally)
that
if
the
head
is
not
dissociated
while
traversing
this
region
(region
III
in
Fig.
8)
that
it
is
mechanically
dissociated
by
the
movement
of
the
filaments,
requiring
an
average
energy
W_.
Using
this
model,
we
can
derive
an
equation
that
describes
the
variation
of
the
maximum
contraction
velocity
with
ATP
concentration.
The
general
force-velocity
relation
may
be
derived
by
writing:
F
V
=
mechanical
power
=
(work
per
interaction)
(cycle
time)-'
(number
of
heads).
(7)
COOKE
AND
BIALEK
Contraction
of
Glycerinated
Muscle
Fibers
249
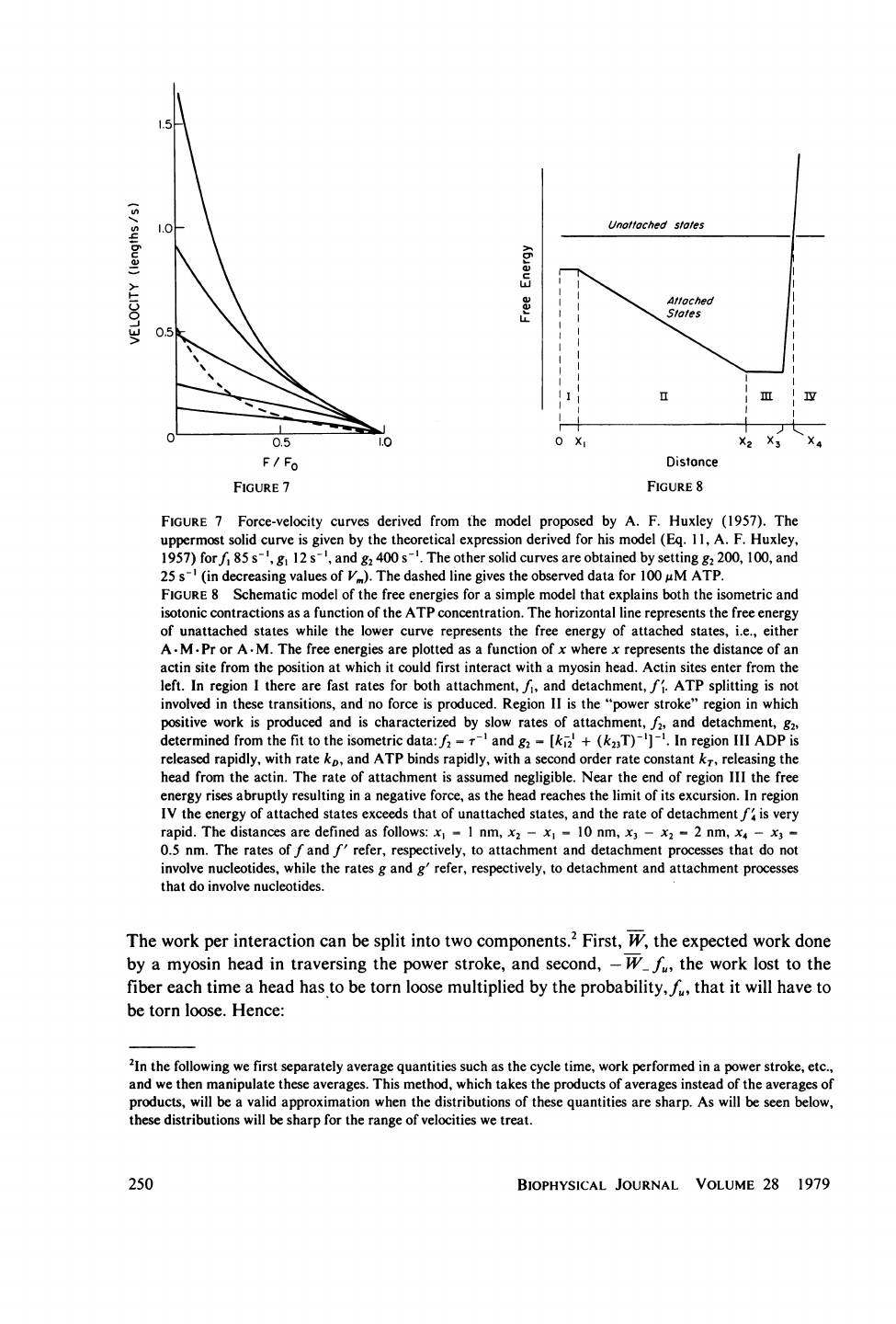
1.0
Unao//ached
stoles
0'
CL
0'5
O'
0.5
1.0
o
xI
X2
X3
X4
F
/
Fo
Distonce
FIGURE
7
FIGURE
8
FIGURE
7
Force-velocity
curves
derived
from
the
model
proposed
by
A.
F.
Huxley
(1957).
The
uppermost
solid
curve
is
given
by
the
theoretical
expression
derived
for
his
model
(Eq.
11,
A.
F.
Huxley,
1957)
forf,
85
s-
',
g1
12
s
',
and
g2
400
s-
'.
The
other
solid
curves
are
obtained
by
setting
g2
200,
100,
and
25
s`
(in
decreasing
values
of
V.).
The
dashed
line
gives
the
observed
data
for
100
,IM
ATP.
FIGURE
8
Schematic
model
of
the
free
energies
for
a
simple
model
that
explains
both
the
isometric
and
isotonic
contractions
as
a
function
of
the
ATP
concentration.
The
horizontal
line
represents
the
free
energy
of
unattached
states
while
the
lower
curve
represents
the
free
energy
of
attached
states,
i.e.,
either
A
.
M
*
Pr
or
A
.
M.
The
free
energies
are
plotted
as
a
function
of
x
where
x
represents
the
distance
of
an
actin
site
from
the
position
at
which
it
could
first
interact
with
a
myosin
head.
Actin
sites
enter
from
the
left.
In
region
I
there
are
fast
rates
for
both
attachment,
f1,
and
detachment,
f'.
ATP
splitting
is
not
involved
in
these
transitions,
and
no
force
is
produced.
Region
II
is
the
"power
stroke"
region
in
which
positive
work
is
produced
and
is
characterized
by
slow
rates
of
attachment,
f2,
and
detachment,
g2,
determined
from
the
fit
to
the
isometric
data:f2
=
r-'
and
g2
=
[k-'
+
(k23T)
'I
'.
In
region
III
ADP
is
released
rapidly,
with
rate
kD,
and
ATP
binds
rapidly,
with
a
second
order
rate
constant
kT,
releasing
the
head from
the
actin.
The
rate
of
attachment
is
assumed
negligible.
Near
the
end
of
region
III
the
free
energy
rises
abruptly
resulting
in
a
negative
force,
as
the
head
reaches
the
limit
of
its
excursion.
In
region
IV
the
energy
of
attached
states
exceeds
that
of
unattached
states,
and
the
rate
of
detachmentf4
is
very
rapid.
The
distances
are
defined
as
follows:
x,
=
I
nm,
x2
-
xI
=
10
nm,
X3
-
X2
=
2
nm,
X4
-
X3=
0.5
nm.
The
rates
off
and
f'
refer,
respectively,
to
attachment
and
detachment
processes
that
do
not
involve
nucleotides,
while
the
rates
g
and
g'
refer,
respectively,
to
detachment
and
attachment
processes
that
do
involve
nucleotides.
The
work
per
interaction
can
be
split
into
two
components.2
First,
W,
the
expected
work
done
by
a
myosin
head
in
traversing
the
power
stroke,
and
second,
-
W_
f,
the
work
lost
to
the
fiber
each
time
a
head
has
to
be
torn
loose
multiplied
by
the
probability,f",
that
it
will
have
to
be
torn
loose.
Hence:
2In
the
following
we
first
separately
average
quantities
such
as
the
cycle
time,
work
performed
in
a
power
stroke,
etc.,
and
we
then
manipulate
these
averages.
This
method,
which
takes
the
products
of
averages
instead
of
the
averages
of
products,
will
be
a
valid
approximation
when
the
distributions
of
these
quantities
are
sharp.
As
will
be
seen
below,
these
distributions
will
be
sharp
for
the
range
of
velocities
we
treat.
BIOPHYSICAL
JOURNAL
VOLUME
28
1979
250

F
*
V
=
[
W
-
W_f,
]
(cycle
time)
-'
(number
of
heads),
where
W,
W
and
f,
are
all
functions
of
velocity
and
ATP
concentration.
Now
we
may
rearrange
to
give:
F(V,
T)
=
[W-
W_f,
]
(X)
-'
(X/
V)
(cycle
time)
-'
(number
of
heads),
(9)
where
X
is
the
expected
distance
traveled
by
a
myosin
head
while
attached
to
actin.
But
X/
V
is
just
the
time
spent
attached;
so
(X/
V)
(cycle
time)-
'
is
the
fraction
of
time
the
myosin
head
spends
attached
to
actin,
or
simply
the
probability
of
being
attached.
Thus
we
have:
F(V,
T)
=
[W-fuW_
]
X-'n(V,
T),
(10)
where
n(
V,
T)
is
the
number
of
attached
heads
at
velocity
V.
Because
neither
n(
V,
T)
nor
X-
can
approach
zero
as
F
approaches
zero,
the
maximum
contraction
velocity,
Vm,
is
determined
by
a
balance
between
heads
exerting
positive
and
negative
forces.
Thus
the
term
in
square
brackets
in
Eq.
10
must
equal
zero
at
Vm.
At
saturating
ATP
concentrations
the
maximum
contraction
velocity
is
determined
by
some
process,
such
as
a
finite
dissociation
rate
for
ADP,
that
is
independent
of
ATP.
However,
at
low
concentrations
of
ATP
the
step
that
determines
the
magnitude
of
fu
is
the
binding
of
ATP.
Since
we
know
from
our
analysis
of
the
isometric
data
that
head
dissociation
is
slow
in
much
of
the
power
stroke,
we
assume
that
rapid
head
dissociation
due
to
ATP
binding
occurs
over
a
distance
AX
near
the
end
of
the
power
stroke
with
a
second
order
rate
constant
kT
(for
the
binding
of
ATP);
we
assume
that
dissociation
occurs
very
rapidly
following
ATP
binding.
If
the
fiber
is
contracting
at
velocity
V,
ATP
has
a
time
At
=
AX/
V
in
which
to
bind,
which
means
that
the
fraction
of
heads
that
are
not
dissociated
by
ATP
is
f
=
exp
(-kT[ATP]At)
=
exp
(-kT[ATP]
AX/V).
At
V,
the
term
in
brackets
in
Eq.
10
is
zero,
and
rearranging
we
have:
Vm
=
[ATP]
{(kTAX)/ln(
/W)1.
(11)
This
expression
gives
a
linear
dependence
of
V,
on
ATP,
as
shown
by
the
data
in
Fig.
5
at
[ATP]
<
200
,uM,
if
the
expression
in
brackets
is
a
constant.
This
expression
will
be
a
constant
if
and
only
if
the
ratio
of
the
positive
and
negative
work
is
a
constant.
The
above
approach
allows
us
to
draw
some
important
conclusions
without
relying
heavily
on
the
details
of
some
model.
Assuming
only
that
head
dissociation
by
the
rapid
binding
of
ATP
occurs
in
a
region
AX
with
rate
kT
we
can
calculate
the
probability
that
a
head
traverses
this
region
undissociated.
Eq.
11
then
allows
us
to
relate
kTAX,
Vm,
and
[ATP]
to
the
unknown
quantities
W.
and
W_,
and
shows
that
their
ratio
evaluated
at
V,
is
a
constant
up
to
a
velocity
of
approximately
1
length/s.
Because
W
and
W_
are
determined
by
different
factors
the
most
obvious
conclusion
is
that
they
are
each
constant.
Although
the
above
does
not
exclude
the
possibility
that
Wand
W_
change
together
in
such
a
way
as
to
keep
their ratio
a
constant,
the
assumption
that
they
do
so
leads
to
unreasonable
models.
As
velocity
increases,
any
change
in
W
will
be
an
increase,
since
heads
torn
off
at
higher
velocities
would
be
expected
to
possess
more
kinetic
energy
after
detachment.
Thus,
W
would
have
to
also
increase.
This
would
require
that
the
force
generated
increase,
since
X
will
not
be
a
strong
function
of
velocity
at
V/m
where
a
large
fraction
of
heads
traverse
the
entire
power
stroke
due
to
a
slow
rate
of
detachment
found
from
the
isometric
data.
Since
it
is
unlikely
that
the
ability
of
a
head
to
generate
force
increases
with
velocity,
the
most
reasonable
assumption
is
that
W
COOKE
AND
BIALEK
Contraction
of
Glycerinated
Muscle
Fibers
(8)
251

and
W_
are
each
independent
of
velocity
up
to
-1
length/s.
If
both
Wand
X
are
independent
of
Vm, then
F,
the
average
force
generated
by
a
head
in
a
power
stroke,
is
also
independent
of
Vyi.
Furthermore,
in
the
above
model,
the
events
that
produce
the
force
are
not
affected
by
the
fact
that
the
muscle
is
contracting
at
Vm;
hence
this
conclusion
can
be
generalized
to
any
velocity,
and
we
conclude
that
F
is
independent
of
the
velocity
of
contraction.
A
Simple
Model
to
Fit
Both
Isometric
and
Isotonic
Data
In
the
above
we
have
analyzed
the
isometric
data
in
terms
of
a
four-state
kinetic
cycle
and
we
have
used
some
simple
energetic
relations
to
draw
conclusions
about
force
production
in
isotonic
contractions.
Together
these
two
approaches
provide
several
constraints
that
more
detailed
models
must
satisfy,
and
in
this
section
we
discuss
these
constraints
and
derive
one
simple
model,
outlined
in
Fig.
8,
which
incorporates
them.
During
an
isometric
contraction
the
low
rate
of
ATPase
demands
that
some
transitions
in
the
cycle
be
slow.
The
data
of
Fig.
2
are
best
explained
by
assuming
a
shift
from
attached
force-producing
states
to
unattached
states
at
ATP
increases.
Thus,
there
must
be
sizeable
fractions
of
both
attached
and
unattached
states
at
high
ATP,
implying
that
both
the
overall
rates
of
detachment
and
attachment
are
slow.
On
the
other
hand,
the
isotonic
data
show
that
the
work
performed
by
each
bridge
is
independent
of
velocity,
which
requires
a
fast
rate
of
attachment.
Thus
the
isotonic
and
isometric
data
must
be
reflecting
rates
of
attachment
and
detachment
of
the
head
in
different
regions
of
head
orientation,
i.e.,
different
values
of
X
in
Fig.
8.
In
fact,
the
apparent
contradiction
can
be
resolved
by
having
a
rapid
rate
of
attachment
preceding
the
power
stroke
(region
1),
and
a
slow
rate
in
the
power
stroke
(region
II).
The
isometric
data
will
be
determined
by
the
events
occurring
in
region
II,
thus
the
rates
in
this
region
(f2
and
g2)
are
determined
from
our
four-state
model
of
isometric
contractions.
In
the
above
model,
heads
attach
rapidly
near
the
beginning
of
the
power
stroke
in
isotonic
contractions,
and,
because
of
the
slow
detachment
rates
in
region
II,
most
heads
will
traverse
its
full
length,
thus
doing
a
constant
amount
of
work.
Obviously,
as
v
approaches
zero
W
must
also
go
to
zero,
and
this
process
begins
as
v
becomes
less
than
g2(X2
-
X,).
We
have
argued
that
at
the
end
of
the
power
stroke
there
is
a
small
region
in
which
rapid
dissociation
of
the
myosin
head
by
ATP
can
take
place.
Rapid
release
of
products
must
also
be
occurring
in
this
region,
since
product
release
is
slow
in
region
II
but
must
precede
ATP
binding.
Thus
there
must
be
fast
rates
for
these
processes
in
a
small
region
(region
III,
assumed,
for
reasons
discussed
later,
to
be
2
nm
long)
that
follows
the
power
stroke.
At
the
end
of
region
III
the
head
comes
to
the
limit
of
its
possible
excursion
and
the
free
energy
increases
rapidly
over
a
short
distance.
When
the
free
energy
of
the
attached
head
exceeds
that
of
an
unattached
head
we
postulate
a
very
rapid
rate
of
detachment
that
does
not
involve
ATP.
Fig.
8
shows
an
energy
level
diagram
of
a
model
that
incorporates
the
constraints
discussed
above.
To
introduce
the
nucleotide
interactions
one
must
have
more
than
two
states.
We
assume
that
there
is
only
one
unattached
state
equivalent
to
M-
Pr,
i.e.,
the
hydrolysis
step,
k34,
is
fast
compared
with
other
rates.
We
assume
that
there
are
two
attached
states,
A.
M.
Pr
and
A
.
M,
which
have
the
same
free
energy,
shown
as
a
function
of
X
(the
position
of
the
actin
relative
to
the
myosin
head)
in
Fig.
8.
The
force
produced
as
a
function
of
velocity
and
ATP
concentration
can
be
calculated
from
the
model
quite
simply
by
methods
outlined
in
the
Appendix.
Force-velocity
curves
were
BIOPHYSICAL
JOURNAL
VOLUME
28
1979
252

TABLE
I
MODEL
PARAMETERS
Determined
from
Determined
from
force-velocity
curves
isometric
tension
(f,
+
f,)
x,
-0.15
s-'lengths'
k,2
=
I
s'
f,
/(f,
+
f)
=
0.55
k23
=
7,000
M'-
s'
kDAX-
1.5s
'lengths
T2
s-
kTAx
=
3,000
M-'
s-'
lengths
W_/W+~=
1.5
Values
of
the
parameters
of
the
model,
outlined
in
Fig.
8,
used
to
fit
the
force-velocity
curves
shown
in
Figs.
3
and
6.
The
three
parameters
in
the
second
column
were
constrained
to
lie
within
the
limits
outlined
in
Eqs.
5.
The
values
of
these
three
parameters,
which
are
chosen
here
to
fit
both
isometric
and
isotonic
data,
do
not
provide
as
good
a
fit
to
the
data
of
Fig.
2
as
do
the
values
given
in
Eq.
3.
In
the
equations
used
to
fit
the
data
some
parameters
can
be
grouped,
e.g.,
in
several
instances
we
fit
the
product
of
a
rate
constant
and
a
length,
and
the
expressions
listed
above
were
the
ones
used.
The
maximum
positive
and
negative
work
in
regions
II
and
III,
W+
and
W_,
are
given
as
follows:
W+
=
F+
(x2
-
x,),
W-
=
F
(X4
-
X3),
where
F+
and
F_
are,
respectively,
the
positive
force
generated
in
region
II
and
the
negative
force
generated
in
regions
III
and
IV.
simulated
on
a
Hewlett-Packard
97
calculator,
and
fit
to
the
data.
The
values
of
the
parameters
that
provided
the
best
fit
of
the
data
are
shown
in
Table
1,
and
the
curves
are
shown
in
Figs.
3
and
6.
The
fit
at
saturating
ATP
concentrations
required
that
(f1
+
f
')XI
be
small
so that
the
fraction
of
attached
heads
decreased
with
velocity
and
the
negative
force
term
was
appreciable
only
at
higher
velocities.
At
low
ATP
concentrations
the
force-velocity
curves
were
determined
by
the
values
of
kTAX
and
W_/
W.
The
value
of
kT
should
be
comparable
to
that
measured
in
solution,
since
the
region
AX
represents
the
region
of
minimum
free
energy
of
the
actomyosin
complex,
and
if
we
assume
AX
2
nm
we
find
kT
-
2
x
106M-
s-
,
in
excellent
agreement
both
with
the
solution
measurement
of
2-4
x
106M-'s-'
(Sleep
and
Taylor,
1976,
White
and
Taylor,
1976)
and
with
our
earlier
conclusion
that
AX
is
small
compared
with
the
power
stroke
(-
10
nm).
The
energy
required
to
break
the
actomyosin
bond
at
the
end
of
the
power
stroke
is
about
1.5
times
greater
than
the
work
produced
in
the
power
stroke.
Approximating
W_
as
the
standard
free
energy
of
an
actomyosin
bond
measured
in
solution
W_-35
kJ/mol,
(for
review,
see
Margossian
and
Lowey,
1978),
W+
is
found
to
be
-25
kJ/mol.
The
maximum
efficiency
of
the
model
is
given
by
W+/AGATP,
where
AGATP
-50
kJ/mol
is
the
free
energy
available
from
ATP
hydrolysis.
Thus,
the
maximum
efficiency
is
-50%,
in
reasonable
agreement
with
physiological
measure-
ments
(Hill,
1938).
We
note
here
that
modifications
of
this
model
discussed
in
the
next
section
will
increase
the
maximum
efficiency
of
the
model.
The
value
of
kDAX
is
not
well
constrained
in
our
model
since
it
plays
an
appreciable
role
only
at
high
velocities
and
high
ATP
concentrations.
The
value
given
has
been
constrained
to
be
too
high
because
in
our
model
the
negative
work
required
to
break
the
actomyosin
bond
does
not
depend
on
the
presence
of
bound
products.
If
this
simplification
is
relaxed
the
value
of
kDAX
will
be
decreased.
Values
off
less
than
103s-
did
not
fit
the
data,
and
the
best
fits
were
obtained
for
f
>
104S-
.
We
have
argued
above
that
the
amount
of
negative
work
performed
by
an
undissociated
head
is
independent
of
the
velocity.
This
will
be
the
case
if
f4
>
V/(X4
-
X3).
Taking
V
=
1
length/s
and
X4
-
X3
=
0.5
nm
=
4
x
10-4
lengths,
we
find
COOKE
AND
BIALEK
Contraction
of
Glycerinated
Muscle
Fibers
253

that
V/(X4
-
X3)
=
2.5
x
IO'
s-l,
and
thus
the
best
fit
of
the
model
to
the
data
again
requires
that
the
negative
work
performed
by
an
undissociated
head
is
a
constant.
The
bond
dissociated
in
region
IV
has
been
distorted
mechanically
so
that
the
equilibrium
constant
for
binding
is
approximately
unity,
a
condition
in
which
very
rapid
dissociation
rates
are
not
unreasonable.
The
model
discussed
above
is
obviously
an
oversimplified
one,
and
its
details
cannot
be
considered
significant.
Rather,
it
is
designed
to
show
that
the
qualitative
conclusions
we
drew
from
the
analysis
of
the
isometric
and
isotonic
data
can
be
incorporated
into
a
single
model
within
the
framework
originally
proposed
by
Huxley
(1957)
and
since
used
by
many
other
workers.
Because
we
have
fit
a
whole
family
of
force-velocity
relations,
we
have
been
able
to
specify
the
rates
for
nucleotide
interactions,
and
have
arrived
at
different
rate
constants
than
those
of
previous
workers.
Although
the
complete
model
of
Fig.
8
may
appear
complex,
its
qualitative
features
are
quite
clear.
Heads
attach
rapidly
at
the
beginning
of
the
power
stroke,
move
through
it
with
almost
no
dissociation,
and
are
dissociated
rapidly
by
ATP
at
the
end
of
the
power
stroke.
Undissociated
bonds
are
then
broken
by
the
motion
of
the
filaments.
Such
a
mechanism
operates
with
high
efficiency
across
a
broad
range
of
velocities,
and
the
rate
of
ATP
hydrolysis
is
tied
in
part
to
the
rate
at
which
work
is
performed,
as
first
observed
by
Fenn
(1923).
Modifications
of
the
Model
In
this
section
we
consider
several
modifications
of
the
simple
model
discussed
above,
beginning
with
the
incorporation
of
thermodynamic
restrictions
that
link
the
kinetic
and
energetic
parameters.
Hill
and
co-workers
have
discussed
the
application
of
these
constraints
to
models
such
as
the
one
above
and
in
the
following
we
shall
follow
their
notation
and
methodology
(Hill
et
al.,
1976).
For
each
ratef
and
g
we
introduce
a
reverse
ratef'
and
g'.
The
rates
are
then
connected
to
the
free
energies
through:
f/f'=
exp
(+A)
g/g
=exp(-A
+
AT),
(12)
where
A
is
the
free
energy
of
unattached
states
minus
that
of
attached
states
and
AT
is
the
free
energy
obtained
from
the
hydrolysis
of
ATP
(free
energies
are
expressed
in
units
of
kT
throughout).
From
the
fit
to
the
data
we
found
that
fi
f,
thus
in
region
I
A
is
approximately
zero.
At
X3
we
approximate
A
as
the
free
energy
required
to
break
an
actomyosin
bond,
A
-
14.
Thus
in
region
II
A
varies
linearly
from
-0
at
X,
to
14
at
X2.
The
back
rate
f'
can
now
be
defined
in
terms
off2
and
Eq.
12
above:
f'2
=
2
exp
[-
14
(X
-
X,)/(X2
-
X1)],
wheref2
=
2
s-
.
Thusf'2
will
decay
rapidly
as
X
increases.
Due
to
the
low
values
off2
andf'2
the
effect
of
fl
will
mainly
be
felt
in
the
number
of
attached
heads
in
region
II
during
an
isometric
contraction,
and
it
will
change
this
number
by
<5%.
Because
AT
is
estimated
to
be
=20,
(Curtin
et
al.,
1974),
which
is
larger
than
A
throughout
regions
II
and
III,
the
back
rate
g2
and
its
equivalent
in
region
III
will
be
negligible.
Thus,
introducing
the
above
rates
will
not
greatly
effect
the
number
of
attached
heads
in
regions
II
and
III.
However,
the
fit
to
the
data
now
requires
that
we
modify
our
view
of
what
happens
in
region
IV.
Using
the
ratio
W
/
W+
=
1.5
and
our
estimate
of
A
at
X3
we
calculate
that
heads
must
be
dissociated
with
BIOPHYSICAL
JOURNAL
VOLUME
28
1979
254

energies
that
exceed
that
of
the
unattached
states
by
-6.
This
can
be
accomplished
by
moving
X4
approximately
0.2
nm
to
the
right
in
Fig.
8.
Takingf
4
=
104s-
as
before,
we
find
thatf4
is
24
s-'
at
X4
and
decreases
rapidly
as
X
increases.
A
simple
calculation
shows
that
the
number
of
attached
heads
once
again
decays
rapidly
with
X
in
region
IV
and
the
net
negative
work
performed
is
still
a
constant.
While
these
modifications
produce
some
further
insights
into
this
model
they
have
not
changed
the
basic
curves
calculated
from
the
more
simple
model
proposed
in
the
section
above.
Certain
additions
to
the
model
could
be
introduced
to
make
it
more
realistic
biochemically.
Among
these
is
the
insertion
of
a
finite
rate
for
the
hydrolysis
step
(or
for
the
transition
from
a
refractory
to
a
nonrefractory
state:
Chock
et
al.,
1976)
that
would
give
rise
to
two
significant
detached
states.
In
addition,
the
states
A.
M
and
A.
M
.Pr
should
be
given
different
free
energies
so
that
there
will
be
a
finite
driving
force
for
product
release.
As
stated
above,
this
would
decrease
our
estimate
of
kD.
Both
of
these
modifications
would
require
considerably
more
data
to
determine
the
new
parameters
they
would
introduce.
Thus,
we
feel
that
the
simple
model
presented
provides
a
satisfactory
physical
explanation
of
our
data.
It
sacrifices
a
more
detailed
and
realistic
framework
but
allows
simplicity
of
calculations
leading
to
insight
into
its
mechanisms.
Relation
to
Other
Work
We
have
worked
with
glycerinated
rabbit
psoas
muscle
fibers
at
10°C,
and
one
question
concerns
whether
our
results
may
be
generalized
to
living
fibers.
At
high
ATP
our
fibers
contract
at
up
to
2
lengths/s,
which
is
close
to
what
one
expects
for
the
living
fiber
at
this
temperature.
The
glycerinated
fibers
exert
-0.1
N/mm2,
which
is
lower
than
found
for
most
living
fibers.
This
is
probably
because
we
worked
at
10°C,
since
at
200C
the
fibers
will
produce
-0.2
N/mm2.
We
chose
to
work
at
the
lower
temperature
because
the
tension
of
the
fibers
is
more
stable
and
the
velocities
are
slow
enough
to
measure.
Data
on
the
ATP
dependence
of
isometric
tension
have
been
taken
on
cardiac
fibers
(Best
et
al.,
1977),
and
on
skeletal
frog
fibers
(Magid,
1974).
Although
the
drop
in
tension
at
[ATP]
<
50
,uM
appears
to
depend
on
the
type
of
preparation
(Kawai
and
Brandt,
1976),
in
all
cases
a
drop
of
-30%
in
tension
is
observed
as
the
ATP
concentration
is
raised
from
50
JIM
up
to
I
mM.
Arata
et
al.
(1977)
also
find
a
maximum
in
the
isometric
tension
of
psoas
fibers
at
20-30
,uM
ATP.
We
know
of
no
published
data
on
the
ATP
dependence
of
the
force-velocity
relation
in
other
muscle
systems;
however,
the
velocity
of
contraction
of
myofibrils
increases
as
ATP
concen-
tration
increases
over
a
wide
range
of
ATP
concentrations
(Arata
et
al.,
1977).
In
addition,
Kawai
(1978)
finds
that
the
frequency
response
of
muscle
fibers,
and
therefore
some
of
the
rate
constants
relating
to
the
velocity
of
contraction,
are
sensitive
to
ATP
at
high
ATP
concentrations
(1-5
mM).
The
frequency
of
oscillation
of
flagella
is
also
dependent
on
the
ATP
concentration
in
a
manner
similar
to
that
observed
here
for
fibers
(Brokaw,
1975),
and
this
process
has
been
modeled
in
some
detail
by
Brokaw
and
Rintala
(1977).
Thus
it
appears
that
the
maximum
velocity
of
both
flagella
and
muscle
is
controlled
up
to
high
substrate
concentration
by
a
similar
rate-limiting
step,
the
binding
of
substrate.
CONCLUSION
When
the
ATP
concentration
inside
a
fiber
is
varied,
it
produces
complex
changes
in
isometric
and
isotonic
contractions.
Our
main
conclusion
is
that
these
changes
can
be
adequately
COOKE
AND
BIALEK
Contraction
of
Glycerinated
Muscle
Fibers
255

explained
by
simple
theories,
consistent
with
the
known
biochemistry
of
the
contractile
interaction,
in
which
the
action
of
ATP
is
to
dissociate
an
actomyosin
bond.
The
isometric
data
can
be
explained
by
a
simple
four-state
model.
We
find
a
rapid
rate
for
the
step
in
which
ATP
binding
dissociates
a
myosin
head
from
actin,
but
the
overall
rate
of
head
dissociation
is
slow,
indicating
that
some
mechanism
operates
to
protect
the
head
from
dissociation.
A
prediction
that
the
ATPase
of
an
isometrically
contracting
fiber
saturates
at
a
higher
level
of
ATP
than
that
measured
for
actomyosin
in
solution
found
experimental
justification
in
the
results
of
Takashi
and
Putnam
(1979).
As
the
ATP
concentration
is
decreased
below
1
mM
the
maximum
contraction
velocity,
Vm,
decreases
dramatically
at
concentrations
where
the
isometric
tension
is
not
greatly
affected.
The
isotonic
data
at
low
ATP
concentrations
indicated
that
rapid
dissociation
of
the
actomyosin
bond
occurs
in
a
small
region
that
follows
the
power
stroke,
with
a
rate
constant
that
is
close
to
that
measured
for
the
analogous
reaction
in
solution.
An
analysis
on
this
basis
concluded
that
the
force
generated
by
a
head
in
a
power
stroke
is
independent
of
the
velocity
of
contraction
(up
to
-1
length/s).
The
above
conclusions
were
incorporated
into
a
simple
model
of
the
actomyosin-nucleotide
interaction,
which
explains
both
isometric
and
isotonic
data.
The
essential
features
of
this
model
are
that
in
an
isotonic
contraction
heads
attach
rapidly
at
the
beginning
of
the
power
stroke,
move
through
it
with
almost
no
dissociation,
and
are
dissociated
rapidly
by
ATP
at
the
end
of
the
power
stroke.
Bonds
not
dissociated
by
ATP
must
be
broken
by
the
motion
of
the
filaments.
We
wish
to
thank
Doctors
Leonard
Peller
and
Evan
Eisenberg
for
helpful
discussions
and
Dr.
Vojtech
Licko
for
help
with
computer
programming.
This
work
was
supported
by
funds
from
U.S.
Public
Health
Service
AM17559
and
AM00479,
National
Science
Foundation
BMS
75-14793,
American
Heart
Association
78-845,
and
a
President's
Undergraduate
Fellowship
from
University
of
California,
Berkeley
(to
Mr.
Bialek).
Receivedfor
publication
19
December
1978
and
in
revisedform
II
May
1979.
APPENDIX
We
here
derive
an
expression
for
the
force
as a
function
of
velocity,
ATP
concentration,
and
the
parameters
of
the
model
outlined
in
Fig.
8.
The
method
is
completely
analogous
to
that
used
by
Huxley
(1957).
Starting
in
region
I,
the
fraction
of
attached
heads,
n(x),
is
related
to
the
rate
constants
by:
Vd
(I
n)fl
-
nf'
(1)
alxI
solving
by
integration
with
the
boundary
condition,
n(O)
=
0,
we
find:
n(x)
=
{1
-
exp
[-(f,
+
f')
x/V]}[f1/(fi
+
f')].
(2)
Using
similar
procedures
we
find
that
the
number
of
attached
heads
in
region
II
relaxes
exponentially
from
n(x,)
to
its
equilibrium
value
f2/(f2
+
g2).
Integrating
from
x,
to
x2
and
dividing
by
the
actin
spacing,
L,
we
find
the
total
number
of
attached
heads
in
region
II:
N11=f2(x2-xX)
V
[
f2f
(2
+
g2)L
f2(x2-XI)
[f2
+
g2
f,
+
f
(
exp
(-(ft
+
fi)x
/
V))][1
-
exp
(-(f2
+
g2)(X2
-
Xl)/v)}.
(3)
BIOPHYSICAL
JOURNAL
VOLUME
28
1979
256

The
positive
force
exerted
will
be
equal
to
F+N11
where
F+
is
the
force
generated
by
each
attached
head
in
region
II.
The
negative
force
will
be
given
by
F-
f
n(x)dx/L.
To
simplify
this
expression
we
assume
that
few
heads
are
dissociated
over
the
small
distance
X3
to
x4
and
thus
the
force
in
this
region
is
F-n(x3)(x4
-
x3)/L.
The
value
of
n
(X3)
is
obtained
by
noting
that
in
region
III
n(x)
decays
from
n(x2)
as
a
result
of
two
processes:
ADP
release,
and
subsequent
ATP
binding
with
dissociation.
Evaluating
the
integral:
x
probability
head
off
at
x
=J
(probability
ADP
released
at
y)
(probability
that
ATP
binds
between
y
and
x)
dy.
(4)
Then
n(x2
)(
1-probability
head
off
at
X3)
we
find:
n(x3)
=
texp
(-kDAX/V)
-
k
DT
k
[exp
(-kTTAX/V)
-
exp
(-kDAX/V)]}
{f2
+
g1
-
i
(
+
f
A
(I
-
exp
(-(f,
+
f')X
/(k))
exp
(-(f2
+
g2)(X2
-
XI)/V)}
(5)
The
negative
force
exerted
in
region
IV
is
given
by:
F_
af
n(x)
dx/L
(6)
which
can
be
evaluated
to
give:
F
n(X3)
V
(7)
The
average
force
generated
by
a
myosin
head
is
now
calculated
from:
F
=
F+n11
-
F-n(x3)(x4
-
x3)/L
-
F-n(x3)V/Lf4.
(8)
We
normalize
our
curves
to
the
force
produced
at
V
=
0:
F
=
(f2/(f2
+
g2)
[(X2-x,
)/L]
F,.
REFERENCES
ARATA,
T.,
L.
MUKOLATA,
and
Y.
TONOMARA.
1977.
Structure
and
function
of
the
two
heads
of
the
myosin
molecule
VI.
J.
Biochem.
(Tokyo).
82:801-812.
BARDEN,
J.,
and
P.
MASON.
1978.
Muscle
cross-bridge
stroke
and
activity
revealed
by
optical
diffraction.
Science
(Wash.
D.C.).
199:1212.
BEST,
P.
M.,
S.
K.
B.
DONALDSON,
and
W.
G.
L.
KERRICK.
1977.
Tension
in
mechanically
disrupted
mammalian
cardiac
cells:
effects
of
magnesium
adenosine
triphosphate.
J.
Physiol.
(Lond.).
265:1.
BROKAW,
C.
J.
1975.
Effects
of
viscosity
and
ATP
concentration
on
the
movement
of
reactivated
sea-urchin
sperm
flagella.
J.
Exp.
Biol.
62:701.
BROKAW,
C.
J.,
and
D.
RINTALA.
1977.
Computer
simulation
of
flagellar
movement
V.
oscillation
of
cross-bridge
models
with
an
ATP-dependent
rate
function.
J.
Mechanochem.
Cell
Motil.
4:205.
CHOCK,
S.,
P.
B.
CHOCK,
and
E.
EISENBERG.
1976.
Pre-steady-state
kinetic
evidence
for
a
cyclic
interaction
of
myosin
subfragment
one
with
actin
during
the
hydrolysis
of
adenosine
5'-triposphate.
Biochemistry.
15:3244.
CIVAN,
M.
M.,
and
R.
J.
PODOLSKY.
1966.
Contraction
kinetics
of
striated
muscle
fibers
following
quick
changes
in
load.
J.
Physiol.
(Lond.).
184:51
1.
COOKE,
R.,
and
K.
E.
FRANKS.
1978.
Generation
of
force
by
single-headed
myosin.
J.
Mol.
Biol.
120:361.
COOKE
AND
BIALEK
Contraction
of
Glycerinated
Muscle
Fibers
257

CROOKS,
R.,
and
R.
COOKE.
1977.
Tension
generation
by
threads
of
contractile
proteins.
J.
Gen.
Physiol.
69:37.
CURTIN,
N.
A.,
C.
GILBERT,
K.
M.
KRETZSCHMARR,
and
D.
R.
WILKIE.
1974.
The
effect
of
the
performance
of
work
on
total
energy
output
and
metabolism
during
muscular
contraction.
J.
Physiol.
(Lond.).
238:455.
EDMAN,
K.
A.
P.,
and
J.
C.
HWANG.
1977.
The
force-velocity
relationship
in
vertebrate
muscle
fibers
at
varied
tonicity
of
the
extracellular
medium.
J.
Physiol.
(Lond.).
269-.255.
EISENBERG,
E.,
and
T.
L.
HILL.
1978.
A
cross-bridge
model
of
muscle
contraction.
Prog.
Biophys.
Mol.
Biol.
33:55.
FENN,
W.
0.
1923.
A
quantitative
comparison
between
the
energy
liberated
and
the
work
performed
by
the
isolated
sartorius
of
the
frog.
J.
Physiol.
(Lond.).
58:175.
FERENCZI,
M.
A.,
Y.
E.
GOLDMAN,
and
R.
M.
SIMMONS.
1979.
The
relation
between
maximum
shortening
velocity
(Vmax)
and
[Mg-ATP]
in
frog
skinned
muscle
fibers.
J.
Physiol.
(Lond.).
292:71.
FERENCZI,
M.
A., E.
HOMSHER,
R.
M.
SIMMONS,
and
D.
R.
TRENTHAM.
1978
Reaction
mechanism
of
the
magnesium
ion-dependent
adenosine
triphosphatase
of
frog
muscle
myosin
and
subfragment-one.
Biochem.
J.
171:165.
FINLAYSON,
B.,
R.
W.
LYMN,
and
E.
W.
TAYLOR.
1969.
Studies
on
the
kinetics
of
formation
and
dissociation
of
the
actomyosin
complex.
Biochemistry.
8:811-819.
GOODNO,
C.
C.
1978.
Kinetic
studies
of
the
myofibrillar
ATPase.
Biophys.
J.
21:3a
(Abstr.).
HILL,
A.
V.
1938.
The
heat
of
shortening
and
the
dynamic
constants
of
muscle.
Proc.
Roy.
Soc.
B.
Biol.
Sci.
126:136.
HILL,
A.
V.
1964.
The
variation
of
total
heat
production
in
a
twitch
with
velocity
of
shortening.
Proc.
Roy.
Soc.
B.
Biol.
Sci.
159:589.
HILL,
T.
L.
1974.
Theoretical
formalism
for
the
sliding
filament
model
of
contraction
of
striated
muscle,
part
I.
Prog.
Biophys.
Mol.
Biol.
28:267.
HILL,
T.
L.,
E.
EISENBERG,
Y.-D.
CHEN,
and
R.
J.
PODOLSKY.
1975.
Some
self-consistent
two-state
sliding
filament
models
of
muscle
contraction.
Biophys
J.
15:335.
HOLT,
J.
C.,
and
S.
LOWEY.
1977.
Distribution
of
alkali
light
chains
in
myosin:
Isolation
of
isoenzymes.
Biochemistry.
16:4398.
HUXLEY,
A.
F.
1957.
Muscle
structure
and
theories
of
contraction.
Prog.
Biophys.
Biophys.
Chem.
7:255.
HUXLEY,
A.
F.,
and
R.
M.
SIMMONS.
1971.
Proposed
mechanism
of
force
generation
in
striated
muscle.
Nature
(Lond.).
223:533.
HUXLEY,
A.
F.,
and
R.
M.
SIMMONS.
1972.
Mechanical
transients
and
the
origin
of
muscular
force.
Cold
Spring
Harbor
Symp.
Quant.
Biol.
37:669.
JULIAN,
F.
J.,
K.
R.
SOLLINS,
and
M.
R.
SOLLINS.
1974.
A
model
for
the
transient
and
steady-state
mechanical
behavior
of
contracting
muscle.
Biophys.
J.
14:546.
KAWAI,
M.
1978.
Head
rotation
or
dissociation.
A
study
of
exponential
rate
processes
in
chemically
skinned
rabbit
muscle
fibers
when
MgATP
concentration
is
changed.
Biophys.
J.
22:97-103.
KAWAI,
M.,
and
P.
W.
BRANDT.
1976.
Two
rigor
states
in
skinned
crayfish
single
muscle
fibers.
J.
Gen.
Physiol.
68:267.
LYMN,
R.
W.
1974.
Actin
activation
of
the
myosin
ATPase:
a
kinetic
analysis.
J.
Theor.
Biol.
43:313.
LYMN,
R.
W.
(1975).
The
steady
state
actomyosin
ATPase:
a
further
note.
J.
Theor.
Biol.
49:425.
LYMN,
R.
W.,
and
E.
W.
TAYLOR.
1971.
Mechanism
of
adenosine
triphosphate
hydrolysis
by
actomyosin.
Biochemistry.
10:
4617.
MAGID,
A.
D.
1974.
The
relationship
of
MgATP
to force
generation
in
striated
muscle.
Ph.D.
Dissertation,
University
of
Washington,
Seattle.
MARGOSSIAN,
S.
S.,
and
S.
LOWEY.
1978.
Interaction
of
myosin
subfragments
with
F-actin.
Biochemistry.
17:5431.
MOOS,
C.
1972.
Actin
activation
of
heavy
meromyosin
and
subfragment-1
ATPases;
steady
state
kinetic
studies.
Cold
Spring
Harbor
Symp.
Quant.
Biol.
37:137.
PODOLSKY,
R.
J.,
and
A.
C.
NOLAN.
1972.
Muscle
contraction,
cross-bridge
kinetics,
and
the
Fenn
effect.
Cold
Spring
Harbor
Symp.
Quant.
Biol.
37:661.
SCHACHAT,
R.
H.,
H.
E.
HARRIS,
and
H.
F.
EPSTEIN.
1977.
Two
homogeneous
myosins
in
body-wall
muscle
of
caenorhabditis
elegans.
Cell.
10:727.
SLEEP,
J.
A.,
and
E.
W.
TAYLOR.
1976.
Intermediate
states
of
actomyosin
adenosine
triphosphatase.
Biochemistry.
15:
5813.
STEIGER,
G.
J.
1977.
Stretch
activation
and
tension
transients
in
cardiac
skeletal
and
insect
flight
muscle.
In
insect
Flight
Muscle.
R.
T.
Tregear,
editor.
Elsevier
Scientific
Publishing
Co.,
Amsterdam.
221.
TAKASHI,
R.,
and
S.
PUTNAM.
1979.
A
fluorimetric
method
for
continuously
assaying
ATPase:
application
to
small
specimens
of
glycerol-extracted
muscle
fibers.
Anal.
Biochem.
92:375.
WHITE,
H.
D.,
and
E.
W.
TAYLOR.
1976.
Energetics
and
mechanism
of
actomyosin
adenosine
triphosphatase.
Biochemistry.
15:5818.
258
BIOPHYSICAL
JOURNAL
VOLUME
28
1979